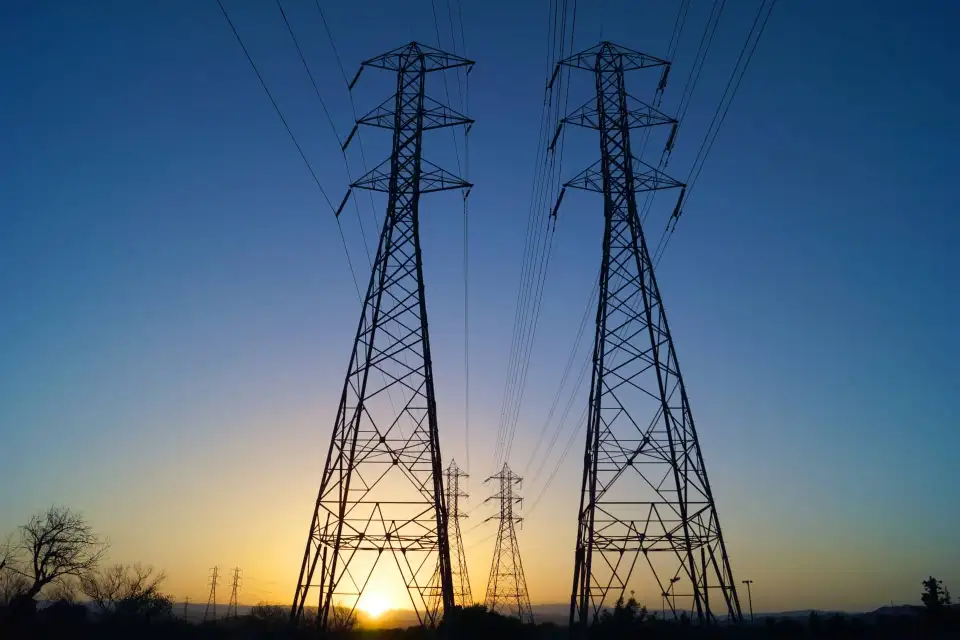
Our world of power and electricity is changing rapidly, from where and how we generate electricity to how we distribute, use, and sell it.
The distribution and transmission infrastructure is aging, the load on the grid is increasing due to electric vehicles and building electrification, and renewable generation resources are coming online at an unprecedented rate. It is estimated that the electric grid will undergo more change in the next decade than it has in the last century. And, building designers, owners, and operators are uniquely positioned to impact how quickly, and costly, the transition to a renewable energy future will be.
Over the last five years, I’ve focused my research at Phius on looking beyond the building: reviewing the existing and changing operations of the electrical grid; the trends, limitations, and challenges confronted within the electricity generation, transmission, and distribution space; and the profound impact our buildings have in that space. I often refer to Phius buildings as the capacitor or critical resource for the new grid (see “Passive Building 101” below). No matter the framing, the core message is that the low-load passive building is critical for facilitating the grid transition away from using fossil-fueled resources to renewable resources (some refer to this as the “Mid-Transition”), and then for resilient operation once we’ve hit that goal (the renewable future). There is a ripple effect from conservation at the building level that perpetuates far beyond the building (see Figure 1).
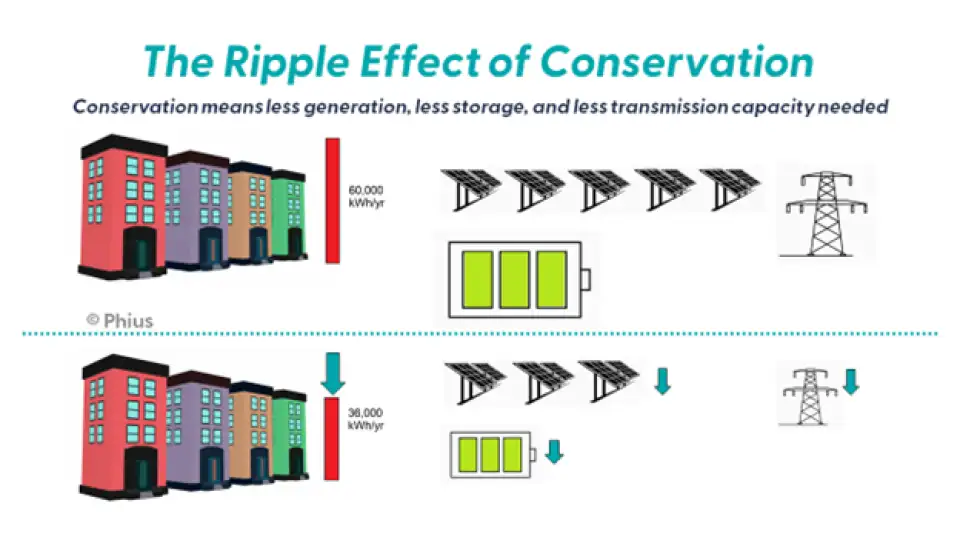
How does a passive building assist this transition to renewable resources? To answer this question, let’s take a closer look at the interdependency between buildings and today’s changing grid (see “Grid 101” below).
The most notable factor reshaping the grid today is that renewable resources are outbidding and replacing dispatchable fossil-fuel based resources. In our existing centralized utility markets, available generation resources bid into the market at the price that they’re able to deliver power, which generally speaking is a combination of operational and fuel costs. Luckily for our push toward decarbonization, wind and solar have no fuel cost and are able to bid at very low prices. Therefore, those are utilized first when possible. As the load increases on the grid, the operators must dispatch more and more expensive resources to meet that load. And often, this is not linear; as the grid load approaches a peak, resource prices may double or triple, which can cause significant spikes in the cost to deliver and purchase electricity during those peak times.
Another important factor affecting the grid is that significant new loads are coming on from electrification of heating, hot water, and cooking loads on top of electric vehicle charging. In areas with substantial heating loads that are currently served by natural gas, the electrification of space heating now places that load on the grid. This change alone will shift the grid peak from the summer to the winter, which is a big deal given that the grid’s generation and transmission capacity was sized in almost all regions to meet a summer peaking load (see Figure 2). The load (and opportunity) presented by electric vehicle charging is a topic for its own article.
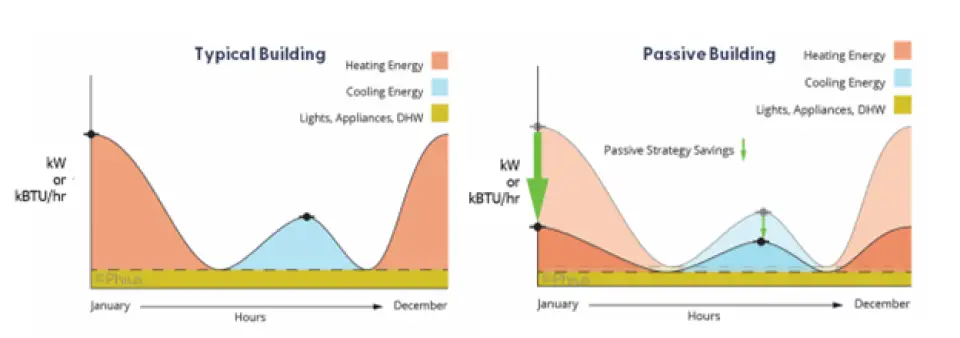
Just as importantly, while the cost to provide electricity is variable, so are the carbon emissions associated with each unit of delivered electricity, and they depend on the mix of generation resources that are utilized to meet the grid load at that given time. Typically, in times of a low grid stress, the emissions are lower, and during high stress, emissions are higher. As more emission-free resources are integrated into the grid mix, there will be a greater disparity between low-emission hours and high-emission hours.
This means that when buildings use energy is becoming increasingly important.
Some grid-regions of the United States are changing more quickly than others, such as California, where renewable energy integration has been accelerated. Areas of higher renewable penetration are experiencing ramping challenges due to renewable resource output steeply entering and exiting the grid mix. They also experience renewable energy curtailment often due to excess production during certain hours (visible through negative energy prices) and due to congestion on the transmission lines (the infrastructure wasn’t sized to carry that load).
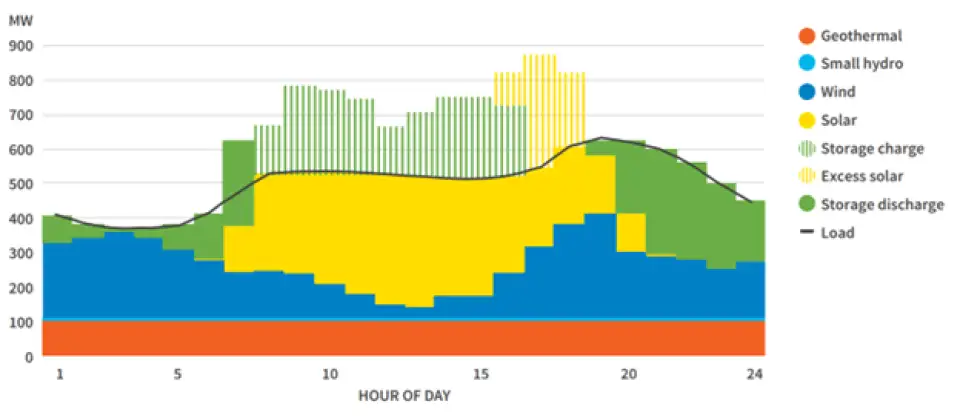
To state it simply, we are intentionally integrating renewable generation resources (supply side) that have generation profiles that do not align identically with our building loads (demand side). In order to fully utilize and continue to rely more on renewable resources we must gain more control of our building load and rely on energy storage to re-shape the profiles into alignment (see Figure 3).
With the grid in mind, let’s get back to passive building and net zero.
Passive building yields low-load, low-emissions buildings. This achievement is already a huge first step for grid friendliness. Remember, the generation resources on the grid are sized to meet the peak load. Passive buildings can dramatically reduce the peak building load, specifically the heating load, often by a factor of two to three compared to a code-compliant counterpart. This translates to two to three times less resource capacity required to match that load. If we consider that fossil-fueled resource capacity is being swapped for renewable capacity, this opens an opportunity to reduce required new capacity.
Passive building load profiles are also flatter, dampening the ramping challenges experienced in areas of high renewable penetration (often referred to as the “duck curve”).
Pairing passive with renewable energy to offset annual energy consumption brings us closer to true decarbonization. This conservation-first approach to net zero is absolutely critical. This is most evident when you consider the resources required on the supply side to meet the demand side. Saving 50% on annual energy use in a building results in the same 50% reduction in the renewable capacity required to offset it, and often this reduction is enough that renewables can now fit on the building’s available roof area. But, this ‘net’ zero concept on its own cannot achieve the ultimate goal of zero emissions.
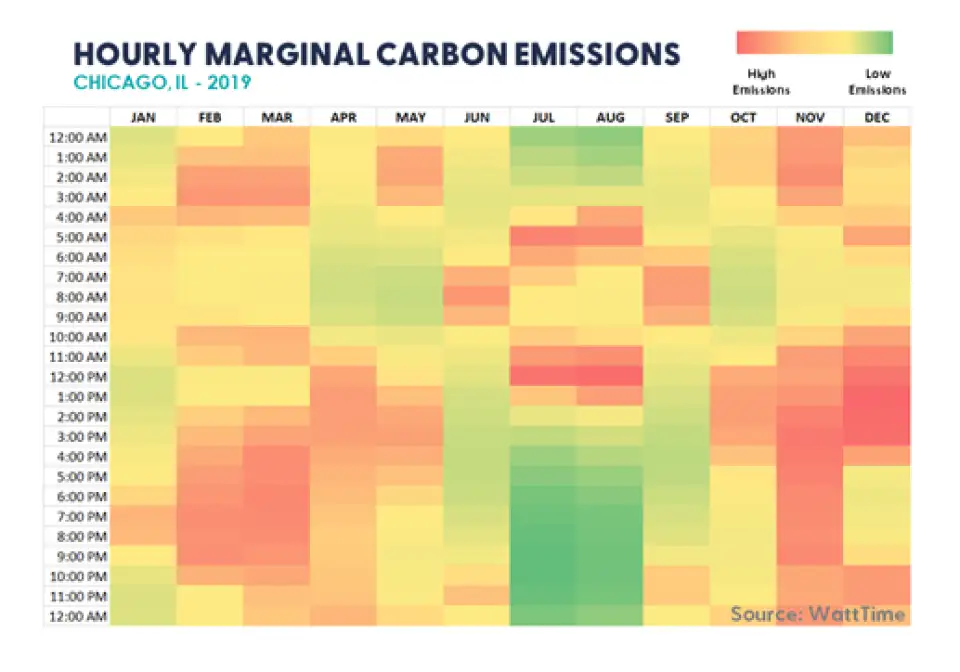
This is where timing comes into play and enabling building loads to align with clean energy supply becomes paramount. Once a building has reached passive levels of reduction in energy consumption, adjusting the timing of energy use may be the next best way to further reduce operational emissions (see Figure 4). Adjusting the load from an hour with high emissions to low emissions could actually reduce the building’s emissions more than continuing to conserve energy or add more renewable energy, depending on when that saved or produced kWh occurred. Aligning building loads with renewable energy availability can also reduce curtailment of excess energy, and if the renewable resource is on or near the building site it can also reduce the stress on the transmission and distribution network.
The importance of timing was well illustrated by Peninsula Clean Energy, a community choice aggregator (CCA) in California, in a recent report it released on its path to provide 24/7 clean energy to its customers by 2025. The CCA already procures 100% renewable energy resources for its customers, but its goal is to deliver 100% renewable energy on a 24/7 basis. The CCA differentiated between these two targets. The “100% clean energy” goal requires purchasing enough renewable energy to cover the customer load over the course of the year, similar to “net zero.” The “24/7 clean energy” means that the CCA must meet the customer load every hour of the year with clean energy, which is a whole different ball game and requires significant coordination and planning between supply and demand.
In the 100% clean energy scenario, renewable energy could be procured that doesn’t align with demand or during times of high renewable output that could be curtailed or otherwise considered less useful.
The reality is, with net zero building, the renewable energy produced or procured isn’t required to align with when the building is using energy. Over the course of the year, it nets out to zero. But over the course of a day, or even seasonally, not so much. Rather than aiming for just “net zero”, I suggest we strive toward “24/7 zero,” which could theoretically be met with a combination of passive levels of efficiency, (super) flexible building loads, renewable energy production and energy storage.
Grid-Interactive technologies are responsible for addressing the timing of energy use and buildings that harness these are referred to as “Grid-Interactive Efficient Buildings” (GEB). Two key GEB technologies are “load shifting” and “load shedding,” also more generally referred to as “demand response”.
Demand response is defined as a building’s change in load based on a grid signal. Historically this has been thought of as reducing load but can also refer to an increase in load. Demand response programs have been around for a while, often targeted at large consumers, such as manufacturing plants. In these programs, consumers are paid to reduce their energy consumption during peak times; it is less expensive for a utility to pay the consumer to use less than it would be for the utility to ramp up more generation. Similar, but less direct, programs are targeted at smaller buildings – sometimes in the form of notifying customers to reduce energy use to avoid spiking prices or in the form of “time-of-use” rates that are generally simplified to “on-peak” and “off-peak” (and my favorite, I recently saw “super off-peak”) pricing.
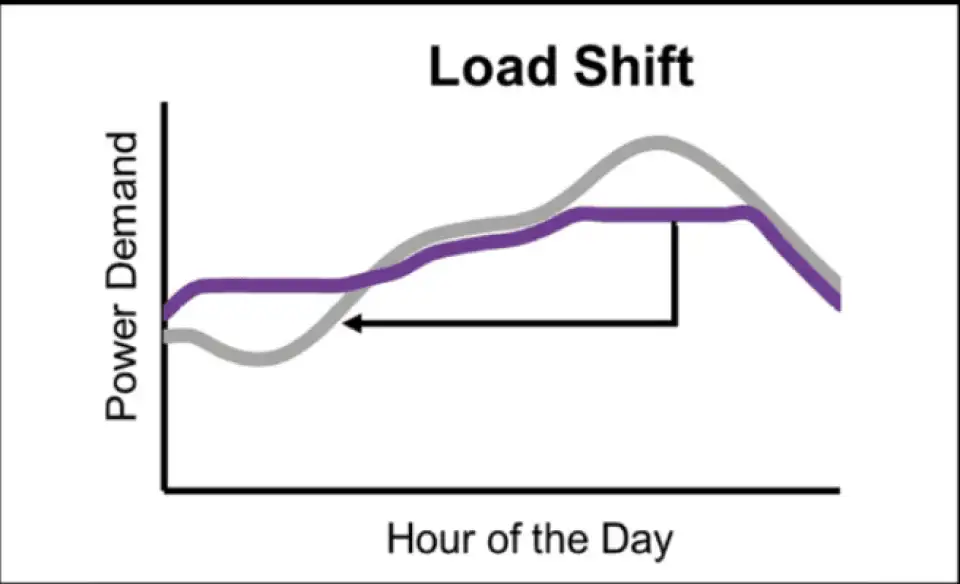
Load shifting can occur with loads that must be met but the timing can be adjusted (see Figure 5). This is most commonly preheating or precooling a space, preheating water or adjusting temperature levels in water heaters, or timing when large appliances are run.
Consider a typical fossil-fueled grid versus a grid with high solar penetration (see Figure 6). In a fossil-fueled grid, the grid emissions are high in the middle of the day when the grid stress is higher. A building connected to that grid would best avoid/shift energy use away from those hours, into the cleaner hours, when possible.
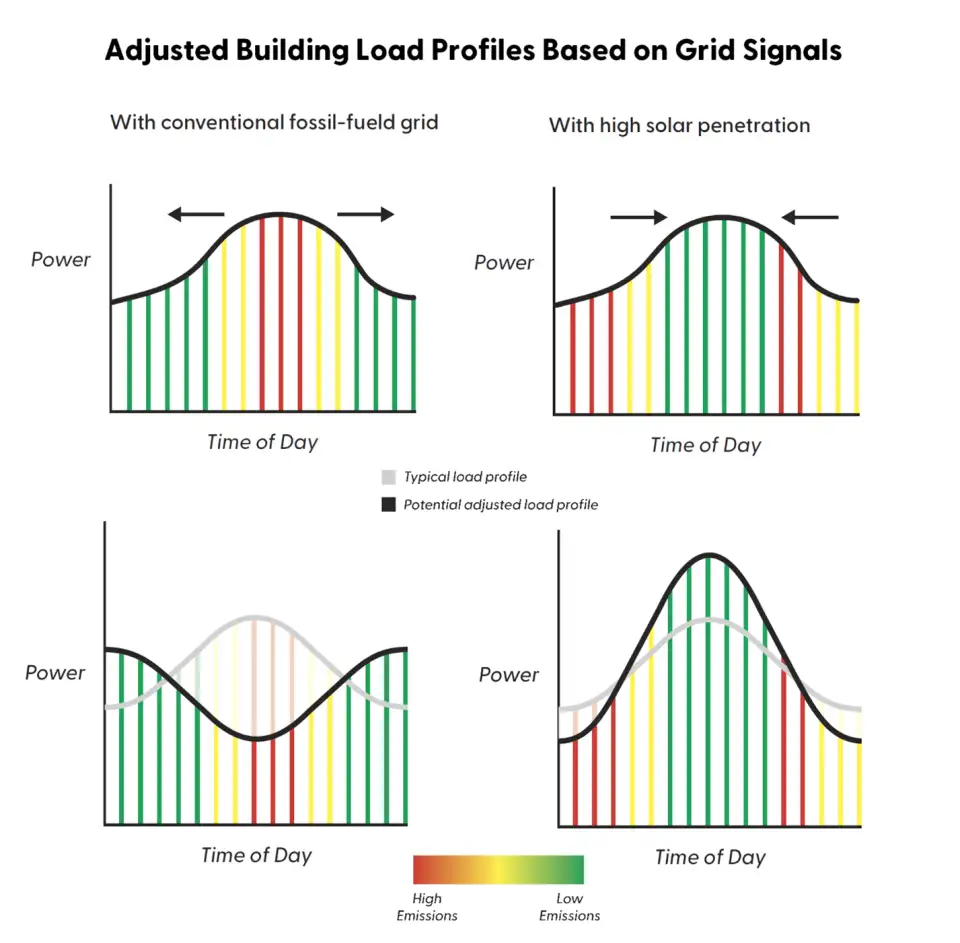
Consider a twin building that has the same load as the building described above, connected to the same fossil-fueled grid but has rooftop solar. Now, the energy resource available in the middle of the day is cleaner than other hours because it is coming directly from the rooftop solar output. That building should be programmed to shift energy use toward the middle of the day, when possible, to align with the cleaner hours.
Load shedding can occur for loads that may be defined as non-critical and can be shed, which most often are space-conditioning loads. When you set your heating setpoint back from 74 to 70, the load shed is the difference between the required output to maintain the higher versus the lower setpoint. This is generally for a short period of time and may be called upon on short notice.
I’ve also recently heard of the “load shimmy,” which refers to quick responses that act as ancillary services for the grid.
Buildings that have significant potential for demand response–load shifting and shedding–are often referred to as those with “load flexibility”. Passive buildings inherently have the potential for load flexibility with space-conditioning loads. Through outage and resilience studies, it has become clear that passive buildings can completely cut space-conditioning system output for significant periods of time (in many cases for multiple hours, depending on the desired indoor condition and the setpoint before any pre-conditioning was used) with little to no impact on the interior temperatures. Their envelopes create a thermal storage system that can be tapped into easily and deeply.
At PhiusCon 2022, Graham Irwin spoke in a lunch keynote about bringing passive building to mainstream market audiences. He joked about how excited he was that “the time constant of passive buildings might be the most untapped resource” (but that wasn’t how or why his clients want to build passive). Anyway, that statement alone was so accurate and simply put that it stuck with me. Just as electrical energy storage paired with renewable resources can make that resource dispatchable and more reliable, thermal storage, made possible through the enhanced thermal enclosure in our buildings, can do the same. And, unlike a battery, we’re already building the building, so why not stretch the benefit?
This load flexibility and shedding capacity can be aggregated between many buildings and called upon in the same way (and at the same price) that traditional generation capacity is dispatched. The ability for a group of buildings to shed load and sell that as supply-side capacity to meet the load can become increasingly valuable—especially if bid in at a high price to be dispatched during peak hours when competing with the most costly resources. Some aggregators do just this, but the volume of participants in demand response programs is low, and the concept still foreign or unsettling to many.
None of this is possible without the appropriate technology and communication protocols between the grid and the building or occupant. These have come a long way over the past decade, with some technology available that can attach directly to existing equipment while other equipment is being sold with grid-enabled technology integrated, allowing that equipment to be programmed to respond to grid signals. In terms of market share, there’s still a way to go. And, there are still challenges with defining the appropriate signals, as well as a lack of access to real-time emissions on the local grid. Occupant participation to configure the appropriate response system for the user is also a hurdle, but it also presents an exciting opportunity for everyone to be part of the renewable energy system transition. On top of this, demand response program managers have reported psychological hurdles of the user not feeling in control. Again, there is a long way to go, but in my opinion, there’s a whole bunch of potential.
Peninsula Clean Energy, the California CCA, is aiming to deliver 24/7 clean energy by 2025. Based on the CCA’s modeling, they could achieve 24/7 clean energy for 99% of the hours in the year with a 2% increase in cost relative to their existing portfolio. But, reaching that last 1% of the hours during cold, winter nights was predicted to increase the required generation procurement capacity by 50%! This is how variable the renewable generation versus demand profiles can be, even in a mild region in California. This suggests we have a real challenge ahead of us.
The next decade is sure to bring innovation and novel solutions to further progress toward a decarbonized economy. I’m incredibly optimistic about the potential of not only passive building, but also grid-interactive efficient building technologies to drive the transformation needed to achieve a fully decarbonized grid and bring a surge of other benefits alongside it.
Passive Building 101
Passive buildings are characterized as buildings that use passive conservation strategies to achieve extremely low loads for heating and cooling and also use significantly less annual energy than a typical building, resulting overall in less carbon emissions.
At Phius, we’ve quantified and defined metrics for passive building. Our passive building standards are rooted in cost-optimization of conservation strategies and set targets for both peak and annual space-conditioning loads. These targets vary based on climate, building size, occupant density, and the cost of delivered electricity. The goal is to reduce total cost (up-front and operational) over time, finding a sweet spot in investment.
We have two main certification tiers, Phius CORE and Phius ZERO. The CORE program targets that sweet spot for efficiency, through passive and active strategies to achieve that low-load building. The Phius ZERO program builds off that to not only target deep efficiency, but also uses a framework that accounts for the produced or procured renewable energy generation in order to net out the building’s energy use on an annual basis. It uses an annual “net” zero concept, meaning the amount of renewable energy produced must be equal to or greater than the amount of energy used in the building on an annual basis. This approach treats all units of energy the same, regardless of when energy is used or produced, which presents a stone left unturned on the path toward building decarbonization.
A main driver of Phius’ building certification program is to decarbonize the built environment. These buildings use conservation strategies to drive down operational energy use, and therefore drive down operational carbon emissions. But we all know that we can’t conserve our way to zero energy use, and, after a certain point, investment in conservation measures may be better spent somewhere else if the true goal is to drive down emissions. After that sweet spot, our program suggests turning to renewable energy offsets to target net zero.
Grid 101
The purpose of the electric grid is to provide power to buildings (and now some cars)—both instantaneously when called upon and uninterruptedly. Grid operators are responsible for ensuring there is sufficient generation resource capacity and sufficient transmission infrastructure to keep buildings running at all hours of the day and days of the year. That is no small feat.
The grid is generally characterized by a supply side and a demand side, with a transmission and distribution network connecting the two. It currently consists mostly of large, centralized generation sources (supply side) that utilize long transmission and distribution networks that carry power to the building loads (demand side). For the most part, it’s run by a one-way communication network—a building load increases and generation output must increase to match that load.
The supply side of the grid is made up of various generation resources and until recently, most of these were fossil-fuel based. These resources vary in terms of dispatchability and generation profiles. Traditional fuel-based power generation such as coal, natural gas, and nuclear are all considered dispatchable, which means that grid operators can control and dispatch them when it is needed to meet the load. But they don’t all have the same level of dispatchability or ramping capability. Coal and nuclear plants are restrained to very small changes in output, while natural gas is well-suited to ramp up and down quickly. Their generation profiles can look fairly similar, but some can be shaped much more easily than others.
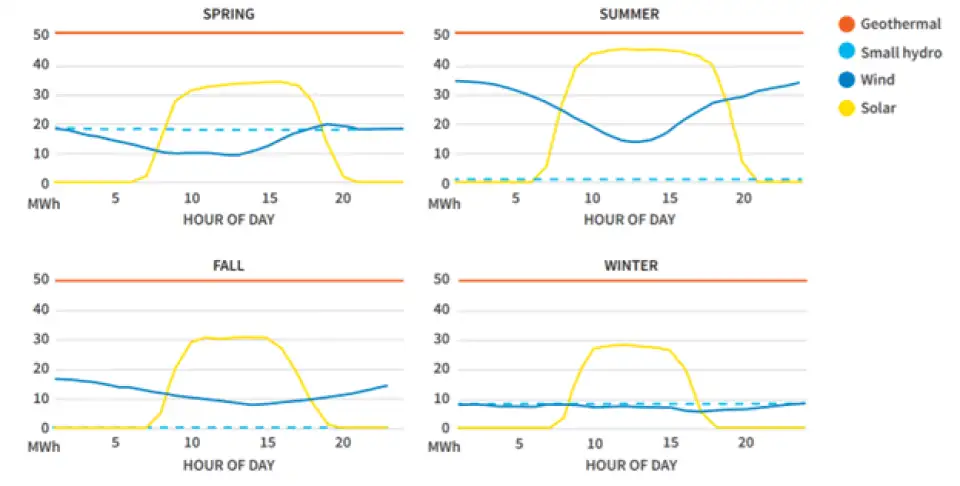
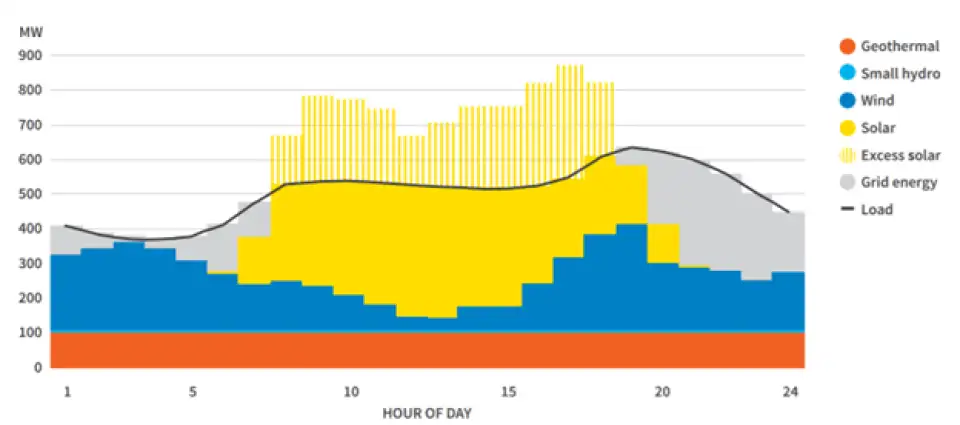
On the other hand, renewable energy resources on their own are not dispatchable. Grid operators cannot tell the sun when to shine, or the wind to blow. But their generation profiles are fairly predictable; we at least know when the sun does or does not have the potential to shine, and have past wind pattern data. These resources also are more distributed rather than centralized (often referred to as distributed energy resources or DER) and may be behind-the-meter, meaning grid operators have no visibility to or control over them, which presents a further challenge. Historically, grid operators have relied on visibility of available resources and have been able to orchestrate dispatching resources as needed to meet the load.
Renewable resources can become dispatchable when paired with energy storage, also allowing their generation profiles to be reshaped to meet the load. In other words, energy storage provides more control over when renewable energy can be used
The profiles I’m referring to in this paper are generation profiles and load profiles, which are often graphically represented as a line chart with hours in the x-axis and power in the y-axis. The top of the curve represents the peak, while the area under the curve represents energy (which is power consumed over time). Generation profiles are those that come from power generation and load profiles are those that result from building energy use.
The installed generation capacity is based on the grid peak and is sized to meet the time where the load on the grid is the highest. Right now, that peak is in the summer in almost all regions of the U.S. due to air conditioning loads. To meet this peak, there’s significant overinvestment in generation capacity for redundancy and reliability. Building designers confront this type of planned redundancy at a smaller scale, such as when the engineer calculates a building’s heating load at a peak, or worst-case design condition, and sizes heating equipment to meet that load and then an extra percentage over, just for good measure. That type of over-sizing and redundancy has been implemented at a much larger scale in the planning and design of the electric grid.
Featured image by Ken Kistler.